The Periodic Table and the Lanthanides
2019 has been designated as the "International Year of the Periodic Table of Chemical Elements" (IYPT2019), celebrating the 150th anniversary of Mendeleev’s discovery of the system (1869).
This is the ninth article to commemorate the “International Year of the Periodic Table of Chemical Elements (IYPT2019)” by the United Nations General Assembly and UNESCO. Read the previous article here and stay tuned
The lanthanide and actinide elements are prominent in the Periodic Table. In the form most usually used, they are conspicuously placed below the bulk of the elements. Readers would have asked their school teacher why and received the following answer: ‘If you study chemistry at University, they will explain that when you get there’. This bulk of elements also make up a sizeable chunk of the table; of the 118 known and named elements, they comprise 15 elements (atomic numbers 57-71), and that is omitting yttrium, which chemically resemble the later lanthanides. How they came to be placed there is a long story; as we will see, Mendeleev did not know where to put them. The lanthanides are often referred to as the rare earth elements. This is an unfortunate misnomer (but so well embedded that we just have to accept it). The least abundant of them, lutetium, is more abundant than silver, gold and the platinum metals (it is about 200 times more abundant than gold, for example). Cerium, the most common, is more abundant than copper.
They do not occur in any concentrated ore, invariably as mixtures with each other, and until relatively recently there have been no easy separations.
The first lanthanide-containing mineral to be identified (at first called ytterbite) was found in 1787, in a small Swedish village called Ytterby. Some of you may think that the name is familiar; maybe that is because this little village has given its name to four of these elements: – ytterbium, yttrium, terbium, and erbium. Back to the mineral, it was named gadolinite in 1800, after Johan Gadolin, the Finnish chemist who discovered the first yttrium compound in 1792. Gadolinite is largely made of the silicates of cerium, lanthanum, neodymium, yttrium, iron, and beryllium; cerium itself was discovered in 1803 by Berzelius and Hisinger and, independently, by Klaproth. It was the first lanthanide to be obtained as an element, by Mosander in 1826. The Swede Carl Gustaf Mosander was a prolific early researcher, credited with the discovery of lanthanum, erbium and terbium, but he did much more than that.
During the 19th century, the discovery of individual lanthanide elements was handicapped by the similarity of neighbouring elements in their chemical properties. All the lanthanides exist primarily as ions with a +3 charge; as they are very similar in size this makes neighbouring lanthanides very difficult to separate from each other. For example in 1843 Carl Mosander separated from lanthanum (newly discovered by Mosander in 1838) a substance that he described as ‘didymium’ (Di), in the belief that it was a new element. It wasn’t. In 1859 two German chemists, Robert Bunsen and Gustav Kirchoff , devised the technique of analytical spectroscopy to identify chemical elements.
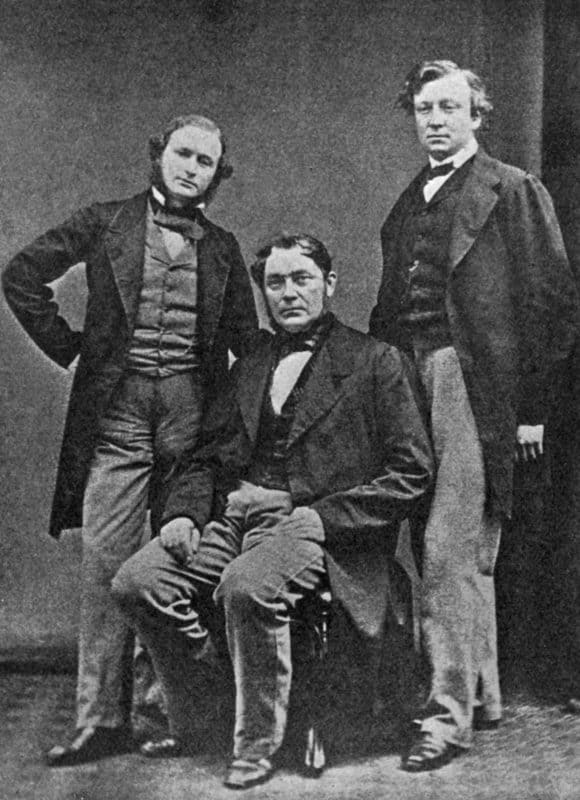
They found that the wavelengths of light emitted by hot atoms of particular elements were characteristic of them, and could be used to identify them. Many will be familiar with the strong yellow light given out by street lamps containing sodium, for example. The following year they were to use it to identify the ‘alkali metals’ rubidium and caesium for the first time). This was to become an important technique in lanthanide chemistry; only six of these elements had been identified in the preceding 60 years yet nine more were discovered in the succeeding half century. Other lanthanides came to be discovered, such as europium, gadolinium, dysprosium, holmium and thulium.
Chemists came to suspect that didymium was not a pure element and in 1879 Lecoq de Boisbaudran separated samarium from the didymium obtained from a mineral sample, and then in 1885 Carl Auer von Welsbach succeeded in making use of the slight solubility difference between two salts, one containing praseodymium and the other neodymium, to obtain these two elements from ‘didymium’. Curiously, ‘didymium’ is still in use today, in safety glasses used by glassblowers and blacksmiths, as it efficiently filters out the intense 589 nm yellow light emitted by hot sodium.
In 1843, Mosander obtained three different ‘earths’ (oxides) of different elements, all obtained from the ore ytterite, coming up with the names yttria, erbia, and terbia (all derived from Ytterby). Subsequently ‘erbia’ yielded ytterbium and erbium, whilst scandium was obtained from gadolinite and in fact the ytterbium was shown in 1907 also to contain the last stable lanthanide to be discovered, lutetium.
Although spectroscopy provided a way of identifying lanthanides, identifying pure lanthanides depended upon separating mixtures, and that in turn depended upon the dexterity and manipulating skills of the chemists concerned. Being able to separate these mixtures by utilising the slightly different solubilities of salts of adjacent metals required many recrystallisations. None could match the patience of the British-born and American-based Charles James, who reported in 1911 that he had carried out 15000 (fifteen thousand) recrystallisations to obtain pure thulium bromate. He was the first person to obtain really pure thulium compounds and reported ‘After about 15,000 operations the absorption spectrum underwent no change.’ A very modest man, James discovered lutetium independently of other workers, but his insistence on checking his results meant that others got into print first.
It was not until the Manhattan project, which speeded up the development of techniques like ion-exchange chromatography, that at last a solution to the problem of lanthanide separations was devised. The lanthanides presented Mendeleev with severe problems in placing them (rephrase). In his 1871 version of the table he placed ‘didymium’ and erbium in Group III and cerium and lanthanum (with an incorrect atomic mass of 180) in Group IV. Yttrium was placed in Group III and a space left in that group for the soon-to-be identified scandium (1879). The increasing number of lanthanides identified through the 1870s and 1880s gave him a read headache.
One of the problems with lanthanide chemistry at the time was that no one knew how precisely how many lanthanides there were. Just after the discovery of lutetium, the answer to this was found by a young researcher, Henry Moseley. He found that when sample of the elements were bombarded with cathode rays, there was a mathematical relationship between the wavelengths of the X-rays that were emitted and the atomic number of the elements.
When the data were put in atomic number sequence, gaps appeared. Moseley showed that there were 15 rare earth elements, one of which (with atomic number 61) still remained to be discovered. Apart from that, from other gaps, he predicted that other missing elements, with atomic numbers 43, 72 and 75 remained to be found; these gaps were filled with the subsequent discovery of technetium, hafnium and rhenium respectively.
Sadly, this was to be Mosley’s one indubitably great scientific discovery. Had he survived, it would assuredly have won him a Nobel Prize. At the outbreak on World War I in 1914 he enlisted in the British Army and joined the Royal Engineers. The following year he was sent to Turkey to join the Gallipoli campaign, and was shot through the head by a sniper at Gallipoli on 10 August 1915. He was 27 years old.
After World War I, a number of investigators took up the quest for element 61 and in 1926 two groups almost simultaneously advanced their claims for the discovery of this element, saying that they had identified its presence in rare-earth mixtures using spectroscopy. Harris, Yntema and Hopkins, who were working at the University of Illinois, suggested the name Illinium (Il), whilst Rolla and Fernandez of the Royal University in Florence, suggested the name florentium. They also claimed to have made this discovery in 1924, but that they had locked their manuscript away in a cupboard for the intervening two years. Bizarre or what?
However other scientists found that known elements could account for the spectral lines assigned to element 61 and the excitement subsided, especially when it was realised that element 61 would have no stable isotopes, that it would be a radioactive element. During World War II, as part of the Manhattan project which lead to the construction of the first nuclear weapons, scientists were studying the elements formed during the nuclear fission of uranium, element 92, which gave rise to smaller atoms. When a uranium atom undergoes fission, it splits into two smaller atoms of unequal size, one of which is usually a lanthanide atom. Marinsky, Glendinin and Coryell, working at the Oak Ridge laboratory in Tennessee, identified an isotope of element 61 among the rare earths formed during the fission of uranium atoms, This discovery was reported in 1947 after it was declassified, and the element was given the name Promethium (Pm). Its most stable isotopes, 145Pm and 147Pm , have half-lives of 17.7 years and 2.6 years respectively, showing that none of the earlier researchers could have detected it in lanthanide ores.
Already by this time it had been decided to place the lanthanide elements separately at the bottom of the Periodic Table. Logically the elements from La to Lu should be accommodated between Groups II and IV, barium and hafnium, but that results in a very long table, certainly one that would prove unwieldy for publishers. Therefore the most usual form of the table places lanthanum below Sc and Y in Group III, with the other lanthanides lined up below the heaviest transition metals in a separate line at the bottom of the table.
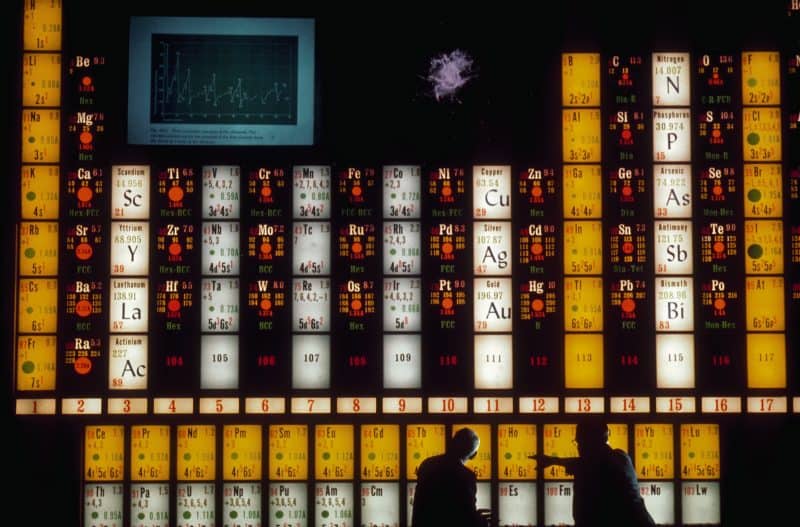
A word about scandium. Because it has no major ores of its own, it tends to be obtained as a by-product of uranium extraction. It fits into the Periodic Table as the first member of the d-block metals, between calcium and titanium. Little studied by chemists, because of its relatively high cost and also because it only exhibits one oxidation state (+3) in the vast majority of its compounds, it nevertheless has some interesting chemistry, not least because it is significantly bigger than the successive d-block metals. Though not a lanthanide, it has tended to be studied at the same time as them. Yttrium is directly below scandium in the table, and significantly bigger. In fact, its size is similar to later lanthanides such as holmium, and its chemistry closely resembles theirs.
But should lanthanum be put into Group III, with elements 58-71 in a block at the bottom of the table? Or should lutetium be placed there, with 57-70 cast adrift? Some 35 years ago, Jensen argued that there were good reasons for the latter approach.
A task group is discussing the matter, with the aim of making recommendations to the International Union for Pure and Applied Chemistry (IUPAC).
The debate continues.
The lanthanides are key to modern technologies, whether it is in phosphors (especially europium and terbium), strong, small permanent magnets (especially neodymium in your car, computer or smartphone) or medicine (e.g. gadolinium in MRI contrast agents).
Who would have guessed what these elements, tucked away at the bottom of the Periodic Table, could get up to?
[Title Image by movieaboutyou on Getty Images ]